For the real amazement, if you wish to be amazed, is this process. You start out as a single cell derived from the coupling of a sperm and an egg; this divides in two, then four, then eight, and so on, and at a certain stage there emerges a single cell which has as all its progeny the human brain. The mere existence of such a cell should be one of the great astonishments of the earth. People ought to be walking around all day, all through their waking hours calling to each other in endless wonderment, talking of nothing except that cell.
Lewis Thomas, The Medusa and the Snail (1979)
Our Approach
Over the past century, scientists have learned an extraordinary amount about the molecular basis of life: how genetic information is stored, copied, and transmitted, and how the instructions contained in DNA are translated into molecules (proteins) that perform a variety of cellular tasks.
What is still lacking, however, is a framework for understanding how this diverse set of proteins and other biomolecules work together to produce functional structures — organelles, cells, and tissues — that are orders of magnitude larger than the size of individual molecules.
In materials physics, continuum theories are commonly used to describe the collective behaviors of systems comprising large numbers of interacting molecules . For over a century, cell biologists have tried to apply these ideas to living structures. Progress has been slow, for at least two reasons: first, measuring material properties in vivo is extremely challenging from an experimental point of view; second, it is not straightforward to apply traditional continuum theories to compositionally complex, far-from-equilibrium living materials. Recent decades, however, have seen significant advances on both fronts: quantitive microscopy facilitates non-invasive characterization of the material properties of cellular structures and progress in active matter physics allows more complete theoretical descriptions of far-from-equilibrium materials.
In our lab, we apply these tools and ideas to better understand two important systems: the meiotic spindle in mammalian oocytes (developing egg cells), and very early mammalian embryos in the process of developing their first distinct tissues. These problems, and how we approach them, are discussed in greater detail in the following sections, but some general principles of our approach are:
- We use microscopes to look at intact structures in living cells
- We leverage the powerful biomolecular tools that have been developed over past decades, and continue to be improved and extended today (molecular biosensors, optogenetics, etc.)
- We apply — and develop/extend where necessary — non-invasive, label-free, quantitive imaging tools (e.g. polarization microscopy)
- We apply — and develop/extend where necessary — conceptual and analytical tools from materials physics and soft matter physics
- We try to understand living structures at the “engineering schematic” level, where molecular details are “coarse-grained” away, but the essential physical properties of the collective are retained. (This is not necessarily always possible or appropriate in biological systems.)
- We look for models that quantitively predict complex biological traits or processes using physcially-motivated models that contain only a small number of measurable parameters.
- We focus on problems, e.g. chromosome segregation in developing oocytes, that are both interesting from a fundamental science perspective and immediately relevant to human health.
Spindles in Mammalian Oocytes
Chromosome segregation is a mechanical process, requiring precisely coordinated motion of micron-sized objects (chromosomes) through distances of tens of microns (the size of a typical metazoan cell). The forces causing this motion are generated by the spindle, a self-organized structure made up of a network of microtubules — long, rigid polymers of the protein tubulin — in association with hundreds of additional proteins that modulate microtubule nucleation, polymerization/depolymerization, and interactions.
Despite our detailed knowledge of the spindle’s molecular constituents, however, as a force-generating mechanical object, it remains very poorly understood.
Our lab uses quantitive imaging techniques, in combination with analytical and conceptual tools from soft matter physics and materials science, to understand the physical principles that underlie spindle assembly and force generation.
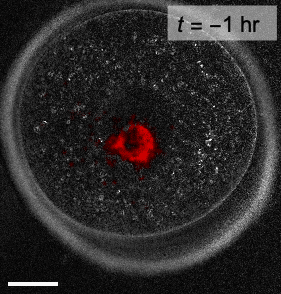
Preimplantation Embryo Mechanics
(details coming soon)
Applications to IVF
(details coming soon)